Introduction
Materials and Methods
Materials
Salicylic Acid and Drought Treatments
Relative Water Content (RWC)
Malondialdehyde (MDA) Content
Total Chlorophyll Content Analysis
Photosynthetic Rate Measurement
Chlorophyll Fluorescence Measurement
Enzyme Extraction for Antioxidant Enzyme Activity Measurement
Catalase (CAT) Activity Assay
Ascorbate Peroxidase (APX) Activity Assay
Glutathione Reductase (GR) Activity Assay
Statistical Analysis
Results and Discussion
The Phenotype and Relative Humidity Depend on the Number of SA Treatments
MDA Content Depends on the Number of SA Treatments
Photosynthetic Integrity Depends on SA Treatment
Changes in Antioxidant Enzyme Activities Depend on SA Treatment
Conclusion
Introduction
Drought stress influences various environmental factors and has been recognized as an critical factor in agricultural productivity (Yang et al., 2010; Sun et al., 2015; Vurukonda et al., 2016; Lee and Park, 2017). In plants exposed to drought stress, transpiration rates are decreased to maintain intracellular humidity levels, resulting in stomata closure. Absorption of CO2, the backbone of photosynthesis, is inhibited by stomata closure, which in turn is activated by abscisic acid (ABA)- mediated regulation of ion flux (Zhu, 2002; He et al., 2012; Khan et al., 2013a). Proton flux is interrupted by inhibiting photosynthesis, resulting in the formation of reactive oxygen species (ROS) (Mignolet-Spruyt et al., 2016). Plants have evolved various methods to reduce the damage incurred by ROS (Ramel et al., 2012). The activation of antioxidant enzymes like catalase (CAT) in the ascorbate-glutathione (ASC-GSH) cycle is essential for the defense response (Kono and Fridovich, 1982; Hernandez et al., 2000). When oxidative stress exceeds antioxidant capacity, ROS accumulate and cause damage to plants (Møller and Sweetlove, 2010). As such, there is a need to better understand the mechanism by which plants utilize stress defense signaling molecules like ethylene, jasmonic acid (JA), salicylic acid (SA), and Ca+ (Swarbreck et al., 2013; Khan and Khan, 2013b).
Among the different signaling molecules in plants, SA is an important molecule that is known to play a role in stress response (Raskin, 1992; Arfan et al., 2007; Lee et al., 2018). SA signaling regulates stress by influencing photosynthesis (Khan et al., 2012). In response to abiotic stress and the accumulation of ROS, SA inhibits the activity of CAT, leading to induction of H2O2 (Kono and Fridovich, 1982). The induced H2O2 is used as a signaling molecule to activate antioxidant mechanisms that reduce damage caused by ROS (Gechev et al., 2002; Møller and Sweetlove, 2010). Researchers have studied the effect of SA on plant growth for several decades (Hayat et al., 2010; Kazemi et al., 2010). However, some reports have demonstrated the negative effects of using high concentrations of exogenous SA (Pancheva et al., 1996; War et al., 2011). Therefore, it is important to determine optimal concentrations of exogenous SA that should be applied and to monitor the duration during which antioxidants are induced.
Chinese cabbage (Brassica rapa L. spp. pekinensis) is an economically important vegetable in Asia (China, Korea, and Japan) (Eom et al., 2018). Chinese cabbage is well known to be a rich source of functional compounds such as calcium, vitamin, carotenoids, free amino acids, proline, glucosinolates, and antioxidants (Wang et al., 2011). Since Chinese cabbage is an important food in Korea, it is especially important to control the drought stress of the seedlings during cultivation (Bray, 1997; Lee and Park, 2017). The control of drought stress in Chinese cabbage seedlings influences the yield and quality of mature leaves (Shawon et al., 2018). The present study reports that exogenous pretreatment with SA can induce antioxidant capacity in Chinese cabbage seedlings.
Materials and Methods
Materials
The Chinese cabbage (Brassica campestris L.) cultivar named ‘Chooseol’ was used in this study. The seedlings of Chinese cabbage were cultivated in a growth chamber (25°C, relative humidity 60%, 12/12h (light/dark), 200 µmol m-2·s-1) for 39 days. Samples (0.1 g) were collected from the upper side of the sixth leaf of seedlings and stored at ‑70°C.
Salicylic Acid and Drought Treatments
Seedlings were treated with salicylic acid at a 0.1 mM final concentration from an aqueous solution. 500 mL of salicylic acid solution was applied to leaves of 23-day-old Chinese cabbage seedlings. For SA treating group 40 pots each. And analysis were conducted in triplicate. Control plants (without salicylic acid) and those treated for three and six times before stopping irrigation were used for the study. Control seedlings were treated with distilled water instead of the salicylic acid solution.
Drought stress was induced after salicylic acid treatment of 28-day-old seedlings by stopping irrigation for 12 days.
Relative Water Content (RWC)
Relative water content was calculated using the following equation (Misra and Dwivedi, 2004).
$$\mathrm{Relative}\;\mathrm{water}\;\mathrm{content}(\%)=(\mathrm{fresh}\;\mathrm{weight}–\mathrm{dry}\;\mathrm{weight})/\mathrm{fresh}\;\mathrm{weight}\times100$$ | (1) |
Malondialdehyde (MDA) Content
0.1 g leaf sample was used to measure MDA content. The degree of lipid peroxidation in plant tissues was measured by applying Metwally’s method (Metwally et al., 2003). The MDA content of plants was quantified following reaction with thiobarbituric acid. Using a mortar and pestle, 5 mL of 0.1% (w/v) trichloroacetic acid was added to the homogenized samples. 2 mL of the homogenized sample was scooped up and centrifuged for 5 minutes at 10,000 g. After centrifugation, 0.4 mL of the supernatant was taken and 1.6 mL of 25% (v/v) trichloroacetic acid was added. The mixture was then heated up to 95°C for 15 minutes, after which the reaction was stopped immediately. After centrifuging the mixture at 10,000 g for 5 minutes, the absorbance of the supernatant was measured at 532 nm and 600 nm using a UV-Visible Spectrophotometer (UV-2450, Shimadzu, Kyoto, Japan). The MDA content was determined using a molar extinction coefficient of 155 mmol-1·L-1·cm-1.
Total Chlorophyll Content Analysis
The content of total chlorophyll in plant tissue was measured using 0.1 g of leaf sample by modifying Arnon’s method (Arnon, 1949). The sample was ground using a mortar and pestle and 10 mL of 80% acetone solution was added. The sample was then wrapped in a foil and stored for 7 days at 4°C in the dark. Next, the absorption coefficient of the chlorophyll extracted acetone solution was measured at 645 nm and 663 nm using a UV-Visible Spectrophotometer (UV-2450, Shimadzu, Kyoto, Japan). The chlorophyll content was determined by using the following equation.
$$\mathrm{Total}\;\mathrm{Chlorophyll}\;(µ\mathrm g/\mathrm{mL})\;=\;20.2\;\times\;\mathrm A645\;+\;8.02\;\times\;\mathrm A663$$ | (2) |
Photosynthetic Rate Measurement
Photosynthetic rates of plant leaves were measured using a Portable Photosynthesis System (LI-6400, Biosciences, USA). Relative humidity was measured as 50 ‑ 60%, CO2 flow rate as 400 mmol/s, chamber temperature at 20°C and light quantity as 200 µmol·m-2·s-1. The upper side of the sixth leaf from a seedling was used to measure photosynthetic rate.
Chlorophyll Fluorescence Measurement
Chlorophyll fluorescence in the leaves of plants was measured using a Chlorophyll Fluorimeter (Pocket Pea; Hansatech, UK). After maintaining the leaves in the dark for 30 minutes, the fluorescence of the leaves, excluding the veins, was measured at 627 nm for 300 µs.
Enzyme Extraction for Antioxidant Enzyme Activity Measurement
2 mL of 25 mM potassium phosphate buffer (pH 7.8) containing 0.4 mM EDTA-4H, 1 mM ascorbic acid, and 2% (w/v) polyvinylpolypyrrolidone were added to a powdered sample and homogenized. The homogenized sample solution was placed in a 2 mL microcentrifuge tube using a micropipette and centrifuged at 15,000 g for 20 minutes. The supernatant obtained was used as a coenzyme extract to measure the antioxidant activities of the enzymes catalase (CAT; EC 1.11.1.6), ascorbate peroxidase (APX; EC 1.11.1.11), and glutathione reductase (GR; EC 1.6.4.2). Total protein was quantified by the Bradford colorimetric method using the coenzyme extract (Bradford, 1976).
Catalase (CAT) Activity Assay
CAT enzyme activity analysis was performed using the method of Aebi (1974). 0.95 mL of the reaction buffer [50 mM potassium phosphate buffer (pH 7.0), 10 mM H2O2] was added to a cubic cell and absorbance at 240 nm was stabilized at 25°C for 30 seconds using a UV-Visible Spectrophotometer (UV-2450, Shimadzu, Kyoto, Japan). 0.05 mL of the crude enzyme extract was then placed in the cubic cell and the change in absorbance was measured for 1 minute.
CAT activity was quantified by the reduction of H2O2 observed upon addition of CAT, and 1 unit was defined as the amount of reduction obtained by 1 µmol enzyme per minute.
Ascorbate Peroxidase (APX) Activity Assay
The analysis of APX enzyme activity was performed as described by Nakano and Asada (1981). 0.94 mL of the reaction buffer [25 mM potassium phosphate buffer (pH 7.0), 0.25 mM AsA (ascorbic acid), 0.1 mM EDTA-4H] was added to a cubic cell, 0.05 mL of the crude enzyme extract was added, and then UV-Visible Spectrophotometer (UV-2450, Shimadzu, Kyoto, Japan) absorbance was stabilized at 290 nm for 30 seconds at 25°C. 10 µL of 10 mM H2O2 was then added to the cubic cell and the change in absorbance was measured for 1 minute.
The activity of APX was calculated as the change in the oxidation rate of AsA used during the reaction of APX, and 1 unit was defined as the amount of reduction obtained by 1 µmol enzyme per minute.
Glutathione Reductase (GR) Activity Assay
GR enzyme activity was analyzed by modifying the method of Foyer and Halliwell (1976). 0.85 ml of the reaction buffer [25 mM potassium phosphate buffer (pH 7.8), 0.06 mM NADPH] was added to a cubic cell and 0.1 mL of the crude enzyme extract was added. It was stabilized at 340 nm for 1 minute 25°C. 10 mM GSSG 0.05 mL was then added in the cubic cell and the change in absorbance was measured for 1 minute.
The activity of GR was calculated as the change in oxidation rate of NADPH used by GR during reaction, and 1 unit was defined as the amount of decrease caused by 1 µmol of reactant per minute.
Statistical Analysis
All experiments and analyses were conducted in a completely randomized design with 3 replicates with individual seedlings for each treatment. Statistical analysis was performed by SAS (version 9.4; SAS Institute, Inc., Cary, NC, USA). Duncan’s multiple range test was used to determine the differences between means (p < 0.05).
Results and Discussion
The Phenotype and Relative Humidity Depend on the Number of SA Treatments
Following 12 days of drought stress, the phenotypes of seedlings were different in response to all treatments. The SA concentration used was established by previous studies, since high concentrations can cause toxicity and low concentrations may not have effects on oxidative stress tolerance (Fariduddin et al., 2003; War et al., 2011; Khan et al., 2015). In the control seedlings, all the leaves were dry, while those treated six times with SA exhibited a phenotype that was less affected by drought stress (Fig. 1). The phenotype depended on the number of treatments and showed an improvement as the number of treatments increased from three to six.
The relative water content (RWC) also showed a significant difference. The highest RWC was observed in the six times treated group, while the lowest was in the control. The RWC started to decrease for all the three groups after six days, and the control rapidly decreased by day 12. SA treatment maintained RWC the most in the six times treated group as compared to the untreated (control) group, while the three times treated group maintained RWC better than the control but less than the six times treated group (Fig. 2). During drought stress conditions, reduced plant RWC is a frequently observed phenotype (Siddique et al., 2000).
In the control, the seedlings started to die under drought stress after 9 days at a death rate of 66.7%. Interestingly, death was delayed and the death rate was decreased by treating with SA. This might be the result of a physiological response to SA that increases alternative pathway under stress conditions in plants (Moynihan et al., 1995). Notably, death did not occur until 12 days in the six times treated seedling group (Table 1). The change in RWC in the plants caused by the drought condition occurred internally, showing a form in which the leaves of the plants sagged. In more severe cases, the leaves withered.
Table 1. Death rate (%) of Chinese cabbage seedlings during drought stress conditions. Seedlings were treated with 0.1 mM SA either three or six times before stopping irrigation. Water was sprayed as a control
DAT (days after drought treatment) | Control | SA treat | |
Three times | Six times | ||
9 | 22.22 ez | 0 g | 0 g |
10 | 33.33 d | 0 g | 0 g |
11 | 41.67 c | 20 f | 0 g |
12 | 66.67 a | 50 b | 0 g |
MDA Content Depends on the Number of SA Treatments
Under drought stress conditions, ROS accumulation results in the oxidation of lipids (Laughton et al., 1989). MDA content is an indicator of plant cell damage caused by lipid peroxidation (Heath and Packer, 1968).
In our study, the untreated control seedlings showed higher MDA content than the other treatments. The MDA content in untreated seedlings increased during all of the drought stress conditions. It slowly increased up to 6 days, and rapidly increased after 6 days. Furthermore, when the Chinese cabbage seedlings were treated with SA, it was found that MDA contents were remarkably low, indicating these plants had less damage than the untreated controls. However, the six times SA treated seedlings had slightly higher MDA contents than the three times SA treated seedlings. This was perhaps due to the fact that SA initially induced H2O2, which quickly decreased (Fig. 3). Overall, six times SA treated seedlings showed the lowest MDA contents after 12 days. These results suggest that pretreatment with SA equips the cabbage seedlings to counter oxidative stress (Belkhadi et al., 2010; Saruhan et al., 2012).
Photosynthetic Integrity Depends on SA Treatment
Plant growth begins with photosynthesis and progresses through a series of primary metabolism processes (Schwachtje and Baldwin, 2008; Detmann et al., 2012). Additionally, photosynthesis has a significant effect on plant growth and leaf yield (Detmann et al., 2012). If photosynthesis in plants is hindered, plant growth can be inhibited because of insufficient CO2 fixation in the stroma of chloroplasts (Schurr et al., 2006; Schwachtje and Baldwin, 2008). Therefore, photosynthesis is important for the growth of plants and plant growth directly depends on the efficiency of the photosynthetic apparatus.
In our present research, we analyzed the photosynthesis rate, chlorophyll fluorescence, and chlorophyll contents. The PI(ABS) value obtained through the measurement of chlorophyll fluorescence indicates the efficiency of the photosystems, the reaction center where photosynthesis occurs (Mathur et al., 2011). We have shown that in untreated seedlings, photosynthetic efficiency was significantly reduced. On the contrary, the groups treated with SA showed about 1.5 times more efficient photosynthesis than the non-treated group, with the six times SA treated seedlings showing the most efficient photosynthesis (Fig. 4A)
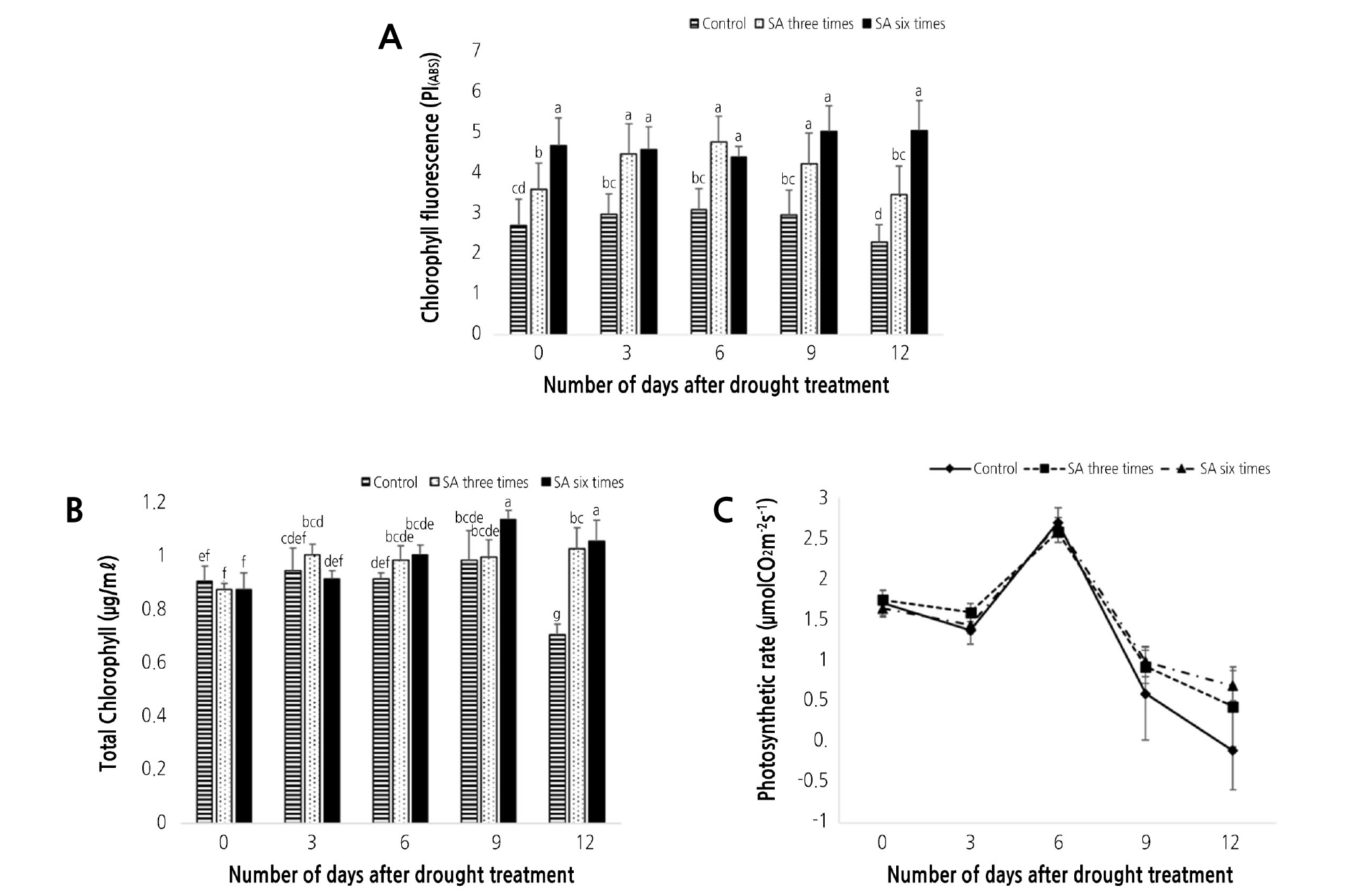
Fig. 4.
The effect of exogenous SA on chlorophyll fluorescence. (A) total chlorophyll content (µg/ml), (B) and photosynthetic rate (µmol CO2 m-2 s-1), (C) in drought condition for 12 days. Error bars indicate ± S.D. of three replicates. Differences in the data indicated by the same letters are not statistically significant (p < 0.05). Control: SA untreated; SA three times: SA treated three times; SA six times: SA treated six times.
The total chlorophyll content was analyzed to determine the capacity to perform photosynthesis (Dai et al., 2009). The chlorophyll content tended to decrease during the 12 days after drought stress condition. When compared to the control, the SA treated group showed a similar tendency to the untreated group up to 9 days, but unlike the untreated group, which had a sharp decrease, the SA treated group contained a steady amount of chlorophyll even on day 12 (Fig. 4B).
Measuring photosynthetic performance and its capacity, we found that the photosynthetic rate decreased by about 10% up to 3 days after irrigation was stopped, after which the rate increased until day 6. After that, the photosynthetic rate drastically decreased. The photosynthesis rate decreased from 6 day after the irrigation was interrupted, and the rate of the untreated SA seedlings converged close to zero. However, the SA treated group showed a higher photosynthetic rate than the untreated group (Fig. 4C).
The photosynthetic performance of the photosystems, as determined by chlorophyll fluorescence, decreased in the untreated SA group (Fig. 4A). A similar trend was also observed in the analysis of the total amount of chlorophyll (Fig. 4B).
The photosynthesis rate, which is highly related to plant growth and is a result of internal physiological activity of plants, started to decrease slightly after irrigation was stopped (Fig. 4C) (Schurr et al., 2006). This is presumed to be the result of ABA-mediated closing of the stoma to prevent water loss under drought stress conditions, due to interrupted irrigation (Miyashita et al., 2005). Later, the photosynthesis rate increased up to 6 days after irrigation was stopped (Fig. 4C). This is presumed to be the mechanism by which plants gain energy through reprogramming, equilibrating, and maximizing the photosynthesis rate after survival under drought stress conditions (Chaves et al., 2009).
Our investigation of the photosynthetic apparatus revealed that SA treatment was effective in maintaining and improving the efficiency of photosynthesis. Furthermore, we showed that when plants were placed under drought stress conditions, they underwent reprogramming to maintain their physiological growth.
Changes in Antioxidant Enzyme Activities Depend on SA Treatment
Plants experience abiotic stress under extreme conditions of water and temperature (Sharma and Dubey, 2005; Hu et al., 2008). Under abiotic stress, ROS accumulate in plants and can damage biomolecules including lipids, proteins, and nucleic acids (Smirnoff, 1993; Munne-Bosch and Penuelas, 2003). Antioxidants counter ROS and thus play a vital role in maintaining homeostasis in plant cells under stress conditions (Kadioglu et al., 2011).
The present study analyzed the antioxidant enzymes catalase (CAT), ascorbate peroxidase (APX), and glutathione deductase (GR), all of which are known to protect plant cells from ROS-induced damage (Møller, 2001). In the absence of SA treatment, CAT activity decreased up to 6 days after stopping irrigation and then increased afterwards. This trend was similar in the two SA treated groups. However, in the treated groups, the extent of decrease in activity was lower and the day of lowest activity was delayed (Fig. 5A).
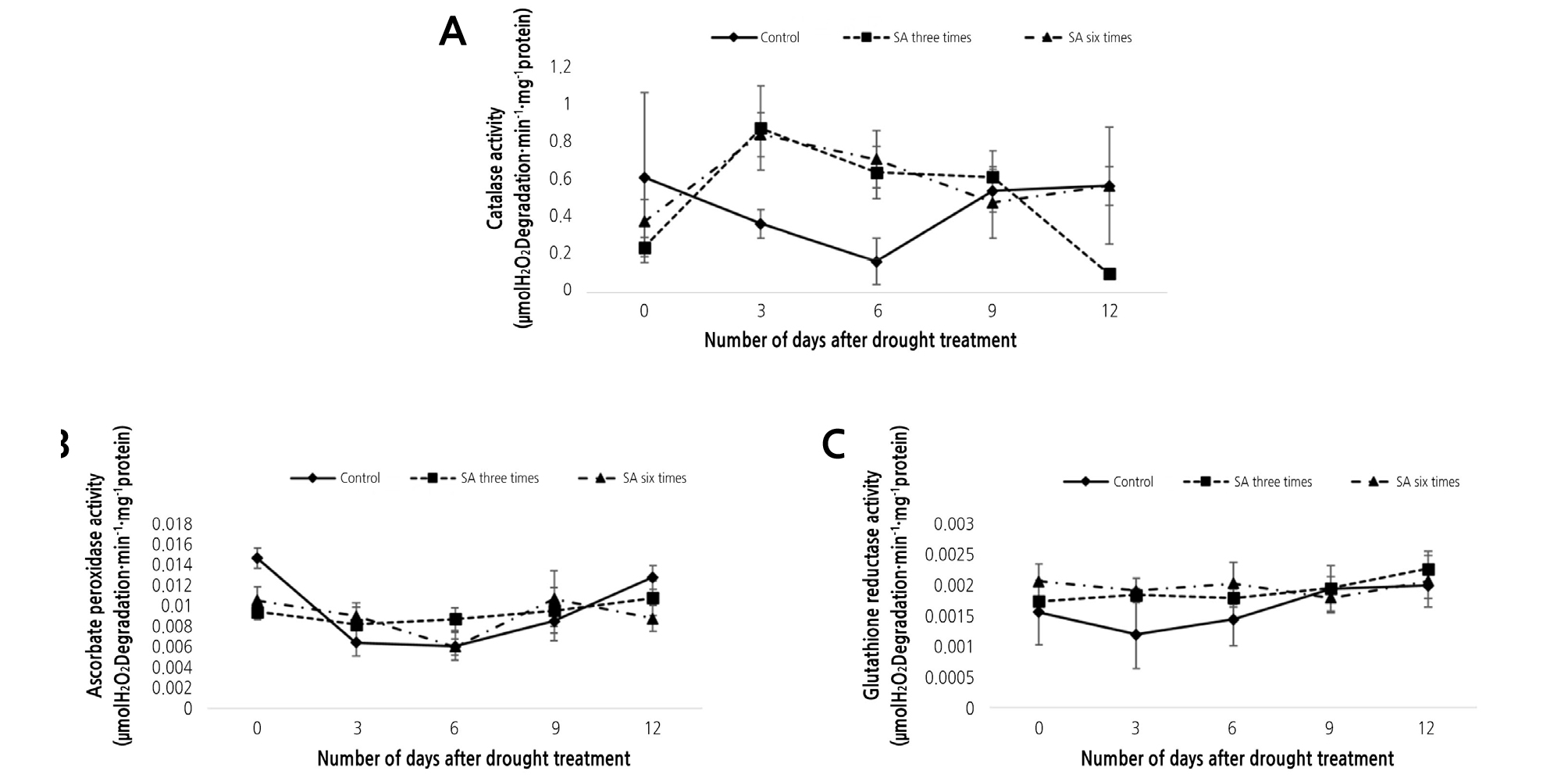
Fig. 5.
The effect of exogenous SA on antioxidant activity related to drought tolerance. (A) catalase activity (µmol H2O2 degradation·min-1·mg protein-1), (B) ascorbate peroxidase activity (µmol H2O2 degradation·min-1·mg protein-1), (C) glutathione reductase activity (µmol NADPH degradation·min-1·mg protein-1) under drought stress conditions for 12 days. Error bars indicate ± S.D. of three replicates. Control: SA untreated; SA three times: SA treated three times; SA six times: SA treated six times.
We observed similar trends for APX and GR activities. In the case of APX, without SA treatment, the enzyme activity decreased until day 3, and then increased afterwards. Following SA treatment, the lowest point of enzyme activity was delayed or not observed. In the case of the three times treated seedlings, the enzyme activity was delayed by 3 days, whereas in the six times treated seedlings, the enzyme activity continued to increase until day 12 (Fig. 5B). In the case of GR, without SA treatment, the enzyme activity decreased up to day 3 and then increased. For the SA treated groups, there were no significant changes in the enzyme activity (Fig. 5C).
Based on previous findings, we believe that the accumulation of ROS due to drought stress results in decreased enzyme activity in the untreated group (Janda et al., 2003). Later on, cellular reprogramming, which increases the antioxidant activity in plants to regain energy in extreme environments (e.g., drought stress), also increases enzyme activity in the untreated group (Chaves et al., 2009; Kocsy et al., 2013).
Additionally, treatment with SA decreased the activity of CAT on day 0. This is presumably because SA initially inhibits the activity of CAT by enhancing the accumulatio of H2O2, which may be used as a signal transduction agent to activate antioxidant mechanisms (Landberg and Greger, 2002; Janda et al., 2003).
Conclusion
The present research reports the benefits of treating Chinese cabbage seedlings, which are affected by drought stress, with SA. Chinese cabbage seedlings are usually transplanted when they are grown for about 3 ‑ 6 weeks. Oftentimes, plants have poor rooting when transplanting, so they can easily be affected by drought stress caused by insufficient water absorption (Sasaki, 2004). Thus, it is very important to manage ROS homeostasis in seedlings during transplantation. In the case of Chinese cabbage seedlings damaged by drought stress, the seedlings displayed decreased relative water content, lower rates of photosynthesis, and enhanced lipid peroxidation. We show that pretreatment of the Chinese cabbage seedlings with SA rescues the negative physiological responses while positively impacting the phenotype of the seedlings. This might be caused by altered signal transduction following exogenous SA treatment (Raskin, 1992). SA plays an important role as a signaling molecule in seedlings, which induces antioxidant activities, alleviates lipid peroxidation, protect the photosynthetic apparatus, and ultimately results in overcoming drought stress. Thus, we propose that SA pretreatment of Chinese cabbage seedlings may be useful as an effective control measure to combat drought stress. In support of this, we demonstrated that the best results were obtained after treating seedlings six times with 0.1 mM SA.